Long COVID, or post‐acute sequelae of coronavirus disease 2019 (PASC), is defined by the World Health Organization (WHO) as the presence of persistent, recurring or de novo symptoms that cannot be attributed to other diagnoses, for more than three months after a severe acute respiratory syndrome coronavirus 2 (SARS‐CoV‐2) infection.1 Based on the limited available data, in 2022, the estimates of PASC in Australia were of 5–10% of coronavirus disease 2019 (COVID‐19) cases,2 whereas global estimates of the prevalence vary from 9% to 81%.3 Recent data from the Victorian Agency for Health Information survey from nearly 13 000 Victorians show that 14.2% of individuals with SARS‐CoV‐2 infection met the definition of having WHO clinical long COVID.4 Variability in these estimates is attributed to differences in research methods, the duration of follow‐up, clinical profiles of study participants, and COVID‐19 severity. For example, the incidence of PASC is estimated to be about 10–30% among patients who were not admitted to hospital,5 increasing to 50–70% among patients with severe and critical COVID‐19 who were hospitalised.6 Based on the above statistics and the documented global number of COVID‐19 cases exceeding 772 million (source WHO, 12 October 2023),7 it is estimated that at least 77 million people worldwide are affected by PASC.1
Although PASC can affect all individuals, the highest percentage of diagnoses is observed among women aged 36–50 years.8 A meta‐analysis that included patients with COVID‐19 admitted to hospital and those treated in the community showed that patients with acute COVID‐19 who required intensive care unit admission and were aged over 40 years were at higher risk of PASC.9 Nevertheless, the reported risk ratio may exhibit a bias favouring studies conducted among severely ill patients with COVID‐19 who were admitted to hospital that are readily accessible for recruitment and follow‐up.
The total reported number of COVID‐19 cases in Australia is more than 11.8 million, with 24 414 (until 25 June 2024) COVID‐19‐related deaths recorded since 2020.10 However, proper national surveillance of cases that continue to experience symptoms beyond three months is lacking and the currently reported PASC prevalence of 5–10% is likely to be an underestimation.
PASC can affect the patients’ physical, mental and emotional wellbeing.11 Along with persistent physical symptoms, such as fatigue, shortness of breath, and pain, the unpredictable nature of the disease can disrupt aspects of life, including social interactions, relationships and work.12 Although the symptoms of PASC are documented to a certain degree, the underlying immunological and physiological factors responsible for the prolonged persistence of these symptoms are largely unknown. The precise mechanisms behind PASC remain the focus of ongoing global research.
Here, we aimed to summarise existing research on PASC, identifying key concepts, gaps, and evidence regarding its pathophysiology and associated clinical manifestations. We included all study designs (human/non‐human) published from January 2020 until September 2023. We conducted searches in PubMed and Web of Science, using specific keywords such as “long COVID”, “post‐acute sequelae of SARS‐CoV‐2”, “PASC” and “chronic COVID syndrome”. Data extraction focused on study characteristics, reported symptoms, diagnostic methods, and pathophysiological mechanisms. We synthesised findings narratively, focusing on major reported physiological symptoms, current clinical trials, and identified gaps for future research.
Multiple biological mechanisms underpinning PASC
Multiple hypotheses have emerged to explain the ongoing symptoms of PASC.13 Among these is immune system dysregulation, which triggers a prolonged systemic inflammatory response that disrupts various organ systems, including the central nervous system. Another proposal is that the prolonged inflammation associated with PASC may be a result of viral reservoirs or persistence of viral particles (eg, in the gastrointestinal tract), or due to a viral reactivation of Epstein–Barr virus (EBV).14 Additional evidence suggests that COVID‐19 triggers immune dysregulation that promotes the generation or expansion of autoantibodies that could promote the initiation of microclots leading to thrombosis.
Dysfunctional neurological signalling
PASC neurological symptoms may arise from direct brain invasion, neuroinflammation or the systemic effects of infection.15 Furthermore, SARS‐CoV‐2‐mediated dysfunctional neurological signalling could lead to dysautonomia and contribute to the peripheral symptoms of PASC.16
SARS‐CoV‐2 has been shown to directly infect brain cells. Infected cells of the choroid plexus, neurons, astrocytes and microglia have been reported using in vitro cell culture and organoid models, brain slice cultures and non‐human primate and rodent models of infection.17,18,19,20,21 A potential consequence of infection could be neuronal and glial cell fusion that has been reported to compromise neuronal activity22 or neuroinflammation. However, neuroinvasion is not required for SARS‐CoV‐2‐induced neuroinflammation.23 A mild respiratory infection in mice, without neuroinvasion, led to prolonged cytokine activity, and microglial activation in the brain which correlated with reduced neurogenesis, oligodendrocyte loss and reduced myelination.23 Similar pathophysiology involving the reactivity of microglia in white matter, shown in both non‐human primates and humans,23,24 can extend the neurological insult from which PASC could arise (Box 1, A).15
In addition, autoimmune‐mediated pathogenesis is a potential candidate for neural dysregulations. A study involving six patients with SARS‐CoV‐2 infection who were admitted to hospital with acute neurological symptoms (eg, encephalopathy, headaches and seizures) used single‐cell transcriptomic analysis of immune cells in blood and cerebrospinal fluid (CSF).18 The study showed that clonal proliferation of specific activated T cells within the CSF was absent in the blood samples. These findings show that T cells and microglial clusters are most highly activated in microglial nodules. Furthermore, differences in the expression of specific immune regulatory molecules between microglial nodules and other anatomical sites suggest that this site‐specific immune infiltration could be a contributing factor to PASC. Another study encompassing 172 patients with moderate and severe COVID‐19 who were admitted to hospital, detected a wide array of serum autoantibodies including those against central nervous system components.25 Cerebral inflammation,26 including the autoantibodies against neuronal and nervous system antigens resulting from SARS‐CoV‐2 infection,27 could potentially serve as a conduit for aberrant signals within the brain, a phenomenon observed in previous research on encephalitic viruses, including the West Nile virus.28 This cascade of inflammation and neuronal dysfunction could potentially have adverse effects on cortical function in severe cases of COVID‐19, which could seed the dysfunctional signalling within and from the central nervous system.
Severe COVID‐19 cases are over‐represented among individuals with reported neural insult, and mild COVID‐19 can also have a significant impact. Elevated biomarkers, such as neurofilament light chain and glial fibrillary acidic protein, indicate ongoing neuronal injury and astrocytic activation in these mild cases.29
Microbiota and metabolism disorders
The high abundance of angiotensin‐converting enzyme 2 (ACE2) receptors in the small intestine30 suggests the capability of intestinal cells to support persistent viral reservoirs,31 a potential mechanism that could contribute to PASC. This gut tropism of SARS‐CoV‐2 correlates with early reported symptoms, including diarrhoea, vomiting and abdominal pain, which may increase the risk of developing gastrointestinal disorders after COVID‐19. These disorders could preferentially arise from pathophysiological changes, including disruptions in the intestinal barrier and imbalances in gut microbiota, leading to mucosal inflammation (Box 1, B).32 This SARS‐CoV‐2‐induced damage of the gut barrier (often referred to as “leaky gut”) results in an increase of bacterial lipopolysaccharide and peptidoglycan at the systemic level,33 including in the kidneys,34 which can amplify systemic inflammation.35 This systemic inflammation is repeatedly observed and reported as the “cytokine storm”, which is also persistent among patients with PASC.36 This “leaky gut” microbiota can generate a range of toxins and uraemic solutes,37 potentially leading to symptoms such as fatigue, disturbances in mineral bone metabolism, neurological issues, and compromised cardiovascular function.
Recent studies have revealed associations between metabolic disorders (eg, type 2 diabetes38 and inflammatory bowel disease39), with alterations in gut microbiota, mucosal inflammation, and increased intestinal permeability after COVID‐19. This increases the risk of metabolic dysfunction, common in PASC, including metabolic dysfunction‐associated fatty liver disease.40 SARS‐CoV‐2 can trigger specific metabolic conditions (eg, metabolic dysfunction‐associated fatty liver disease) and can push a compromised system into a positive feedback loop, even contributing to limited mitochondrial function.41 In addition, SARS‐CoV‐2 is reported to morphologically modify infected cells with noticeably thinner mitochondria congregating around double‐stranded RNA‐containing vesicles42 and unusual swelling in their mitochondrial cristae.43 These infected cells also downregulate crucial metabolic genes responsible for the expression of antioxidants and respiratory chain proteins,44 affecting oxidative phosphorylation44 and mitochondrial calcium sequestration,45 thereby affecting cellular signalling cascades. Similar mitochondrial dysfunction has been associated with myalgic encephalomyelitis/chronic fatigue syndrome (ME/CFS),46 and may result from the reactivation of EBV.47
Immunothrombosis
Immunothrombosis refers to a complex interaction between the immune and haemostatic systems. It involves the formation of blood clots (thrombi) because of immune responses and inflammation, rather than just traditional clotting mechanisms. The phenomenon of immunothrombosis involves several elements: endothelial inflammation, formation of microthrombi, and the disruption of connections between endothelial cells.48 Given the interconnectedness of the immune and blood clotting (haemostatic) systems, various factors have been implicated in triggering immunothrombosis in PASC.49 Among them, elevated cytokine levels during the infection and activated platelets have been associated with coagulation abnormalities in the context of PASC (Box 1, C),50 fostering a proinflammatory environment affecting the microvasculature.51
Central to the compromised microvasculature are dysfunctional endothelial cells, contributing to the increased clotting events observed in both COVID‐19 and PASC.50 Furthermore, under inflammatory conditions, endothelial cells release tissue factor, which, in the context of COVID‐19, triggers the activation of the coagulation cascade upon entering the bloodstream.52 This demonstrated evidence highlights the presence of impaired perfusion and endothelial dysfunction in COVID‐19,53 which may contribute to the extensive visceral multiorgan and neurological manifestations of the disease.54 This dysfunction could be sustained in PASC cases where the continual release of spike protein55 can potentially downregulate the ACE2 receptor.56 The activation of pericytes contributes to the secretion of proinflammatory molecules typically involved in hypercytokinaemia, further inducing proapoptotic factors that cause endothelial cell death.57 A comprehensive study revealed that endothelial dysfunction independently stands as a risk factor for PASC where endothelial biomarkers such as endothelin 1 (ET‐1) were significantly higher in both patients with ME/CFS and patients with PASC compared with healthy donors.58 These findings indicate a connection between the SARS‐CoV‐2 infection‐mediated rise in the biomarker ET‐1 that is associated with vasoconstriction,59 with the potential to lead to endothelial dysfunction in both severe COVID‐1959 and PASC.60
Taken together, these frequent occurrences of endothelial dysfunction have prompted researchers to view COVID‐19 not only as a respiratory illness but also as a vascular disorder.
Immune dysregulation
A recurring observation in PASC is the continued activation of primary immune cells like neutrophils, monocytes and mast cells (Box 1, D). Research conducted during the subacute phase after SARS‐CoV‐2 infection indicates that the continued activation of granulocytes such as neutrophils plays a crucial role in determining disease severity,61 and that they can persist for an extended period.62 These include heightened levels of neutrophil‐mediated NETosis induction (triggers the release of neutrophil extracellular traps [NETs]), which has been valuable in distinguishing disease severity and patients with PASC.63 The symptoms resembling those of mast cell activation syndrome are amplified in PASC cases64 and thus it is plausible that augmented activation of malfunctioning mast cells65 contributes to the pathophysiology in PASC. Furthermore, studies have reported an expanded population of CD14+ and CD16+ intermediate monocytes and activated CD38+ HLA‐DR+ myeloid cells for up to eight to 15 months following mild to moderate COVID‐19.66 Given the relatively short lifespan of circulating innate immune effector cells such as neutrophils and monocytes, this raises questions regarding the potential modulation of hematopoietic progenitor cells in the bone marrow after SARS‐CoV‐2 infection, potentially contributing to sustained inflammation and hyperactivity of these innate immune cells.
The persistent activation of adaptive immune cells has been observed in PASC,67,68 potentially contributing to inflammation‐associated symptoms. These phenomena are supported by studies demonstrating that individuals with PASC exhibit a marked decrease in naïve T and B cells over time, alongside the persistent highly activated state of innate immune cells. Moreover, elevated serum levels of type I interferon (IFN‐β) and type III IFN (IFN‐λ1)69 are consistently reported in these cases in the context of post‐COVID‐19 complications.67,68
These systemic dysfunctions are increasingly evident, as individuals with acute severe COVID‐19 exhibit increased exhausted/senescent T cells, which persists into their convalescent phase, where disruptions in the population of CD4+ T regulatory cells become apparent.70 This aberrant lymphocyte population, combined with the immunosuppressant administered to patients with COVID‐19, has been attributed to cases of viral reactivation, specifically EBV and cytomegalovirus, presenting another hypothesis for PASC pathophysiology.71 If the persistence of SARS‐CoV‐2 is established, it could lead to a continuous activation of the adaptive immune system, creating a chronically inflamed environment. Additionally, CD8+ T cells in patients with PASC displayed a significant increase in CD57+ terminal effector cell phenotype/subpopulations.70 Moreover, persistent activation of T cells, unresolved inflammation and cytokine storm, persists in severe COVID‐19 cases up to one year after infection.72 These elevated cytokine markers in severe COVID‐19 may enhance T cell responsiveness to interleukin‐15‐driven bystander activation.73 Consequently, the low baseline levels of anti‐SARS‐CoV‐2 IgG levels74 during acute COVID‐19 predict the likelihood of experiencing PASC symptoms six to seven months later, regardless of hospitalisation status.75
PASC is associated with multiple immune dysfunctions. However, further research is needed to clarify the role and impact of each system on the persistence of symptoms, enabling the development of tailored solutions.
Autoimmunity
Ongoing research to understand PASC suggests that SARS‐CoV‐2 infection may trigger autoimmune responses in (potentially genetically predisposed) individuals.76,77 One prominent autoimmune condition reported among individuals living with PASC is onset of diabetes, which proposes that increased β‐cell autoantibodies might play a role (Box 1, E).78 Amid differing autoimmune theories, alternative non‐autoimmune evidence suggests that SARS‐CoV‐2 can infiltrate pancreatic cells where destruction of insulin‐producing β‐cells may cause a marked decrease in insulin levels,79 and thus disturbances in glycaemic control.77 Furthermore, studies have shown a high occurrence of diabetic ketoacidosis in individuals with COVID‐19‐induced diabetes,38 suggesting a possibility of acute viral‐induced damage to the pancreas that cannot be disregarded.
Conversely, research has demonstrated that PASC‐associated inflammation, via the production of autoantibodies, can trigger autoimmune conditions such as arthritis80 and systemic lupus erythematosus (SLE).81 Although the precise mechanism remains unclear, there is speculation that factors such as type I IFN signatures and skewed B cell populations, including expanded extrafollicular B cells driving short‐lived antibody responses, or atypical memory B cells, previously described in SLE and other autoimmune contexts, may play a role.82,83 There is evidence that the expansion of autoantibody against type I IFN is a significant cause of mortality among patients with COVID‐19,84,85 but it is unclear whether this plays a role in PASC. A recent study showed a negative correlation between anti‐SARS‐CoV‐2 IgG and anti‐IFN‐α2 antibodies (Box 1, F).86 This raises two areas for investigation, first it prompts consideration of whether anti‐IFN‐α2 antibodies could interfere with IFN‐α2 signalling, thereby disrupting the IFN‐dependent B cell response and reducing the production of virus‐specific antibodies while enhancing autoantibody production. Second, inhibiting IFN‐α2 may lead to an increase in pro‐inflammatory cytokines and associated cell damage, which could stimulate the development of antinuclear antibodies targeting self‐antigens. Studies have shown that more than 70% of patients with severe acute COVID‐19 exhibit systemic autoimmune rheumatic disease‐associated autoantibodies, including antinuclear antibodies, anticardiolipin, and anti‐β2‐glycoprotein‐1. This suggests that systemic autoimmune rheumatic disease may be a PASC complication.88 Henceforth, it is crucial for forthcoming research to underscore the potential role of SARS‐CoV‐2 infection as a trigger in individuals with a pre‐existing predisposition to autoimmune disorders, emphasising the need for inclusive representation in patient cohorts.
Conclusion
In the evolving landscape of COVID‐19, fundamental insights into the complex interplay of interconnected mechanisms are emerging that underlie the persistence of symptoms long after clearance of the initial SARS‐CoV‐2 infection. Although there is growing consensus regarding the symptoms that could be attributed to PASC,89 there remains a lack of diagnostic tests, limited treatment choices, no proven effective treatments (Box 2), and an incomplete understanding of its prevalence. Many of the proposed mechanisms, and symptoms, are not unique to SARS‐CoV‐2 infection, but share features with other severe viral infection, which can perturb multiple biological systems and lead to post‐infection sequelae in patients. However, there is the limited number of control studies in humans with other common respiratory illnesses resulting in severe disease outcomes. Hence, extensive research is urgently required to address these gaps (Box 3).
Immune dysregulation is a central theme, marked by the sustained activation of primary immune cells, exhaustion and/or depletion of T cells, skewed B cell profiles and disruptions in adaptive immunity crosstalk. The gut environment is emerging as a nexus between microbiota, metabolism, and systemic dysfunction, potentially sharing pathophysiology mechanisms with conditions such as ME/CFS. We believe that research into PASC, informed by the clearer context of post‐COVID‐19, holds significant potential to elucidate mechanisms that underlie ME/CFS. This cross‐disciplinary approach could ultimately benefit patients with post‐infection sequalae. Autoimmunity arises as a significant factor, as evidenced by the development of autoimmune disorders and the detection of autoantibodies and other autoimmune‐associated post‐COVID‐19 complications. Immunothrombosis underscores the interplay between the immune and haemostatic systems, while dysfunctional neurological signalling highlights the far‐reaching impact of SARS‐CoV‐2 infection on the central nervous system.
This multifaceted picture underscores the complexity of PASC, which extends well beyond acute infection. This further emphasises the critical need for a holistic and comprehensive understanding of the mechanisms underlying PASC to design precise diagnostic tools and targeted interventions to alleviate the enduring burdens of this chronic condition. It is evident that PASC is not a single disease, but a spectrum of post‐acute sequelae; therefore, no single mechanism will account for the diverse manifestations falling under the PASC umbrella diagnosis. Instead, multiple mechanisms are likely responsible for various presentations of this condition, underpinning the broad range of symptoms.
With ongoing and dedicated research, we anticipate the identification of biomarkers that will enhance the clinical diagnosis and classification of patients with PASC. Global and multidisciplinary research efforts offer great promise for expediting the discovery of new therapeutic targets, providing hope for effective treatment options shortly.
Box 1 – Proposed mechanisms for post‐acute sequelae of coronavirus disease 2019 (PASC) pathogenesis
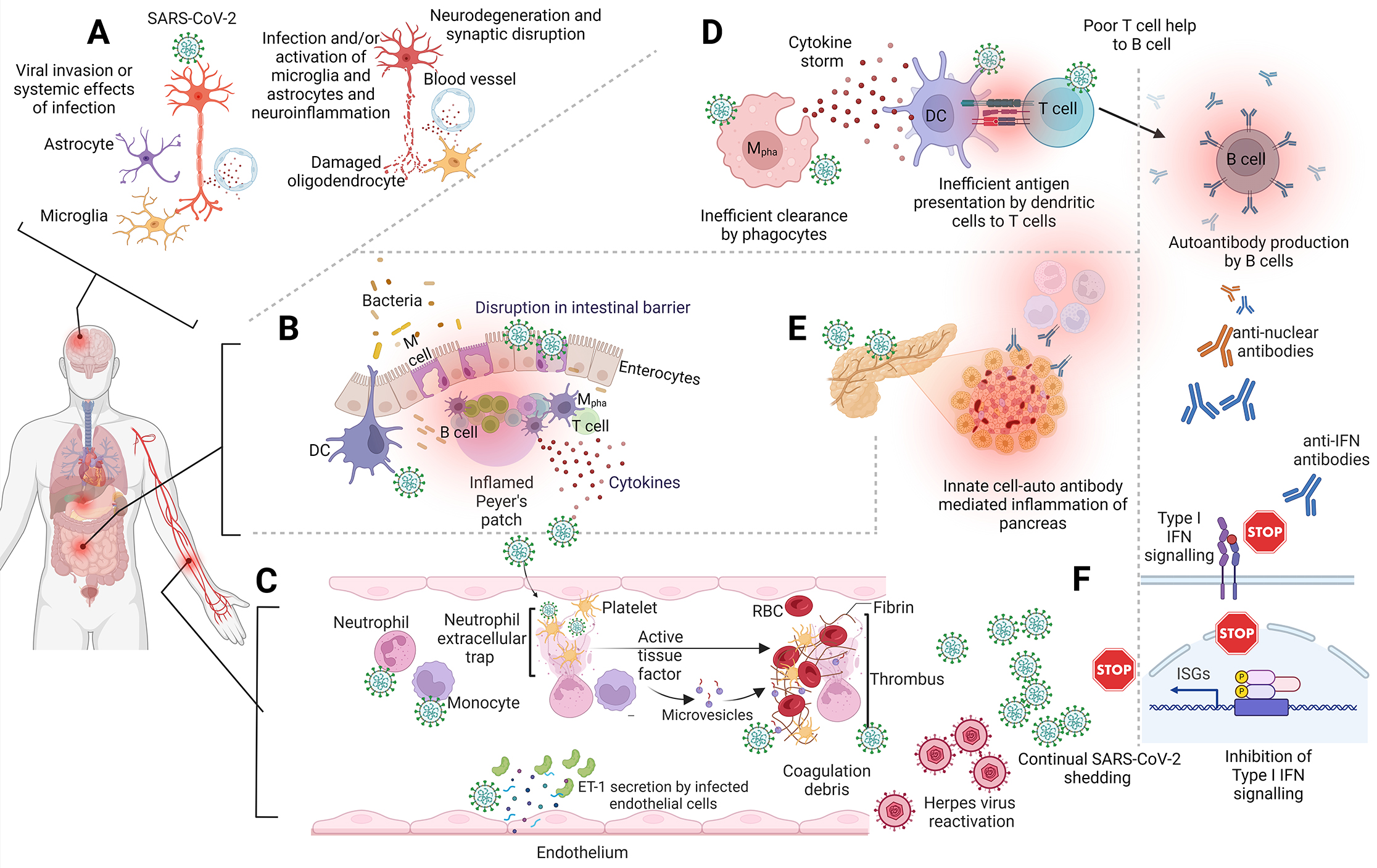
ET‐1 = endothelin 1; DC = dendritic cells; IFN = interferon; ISG = interferon‐stimulated genes; M = membranous cell; Mpha = macrophage; RBC = red blood cell; SARS‐CoV‐2 = severe acute respiratory disease coronavirus 2. (A) The invasion of SARS‐CoV‐2 or the systemic effects of infection triggers microglia and astrocytes to generate neuroinflammation, leading to the impairment of oligodendrocytes, ultimately causing neurodegeneration and disruption of synaptic function. (B) Potential damaged gut/intestinal barrier allowing commensal bacteria into the visceral tissues leading to an inflamed gut blood axis inflaming the Peyer patch. (C) The hyperactivation of circulatory neutrophils and monocytes promotes the damage of endothelial linings within blood vessels by direct infection, which is characterised by increased expression of vasoconstrictors such as ET‐1. This can activate the complement and platelet‐associated tissue coagulation factors increasing the incidence of coagulation debris. (D) Immune dysregulation spanning circulatory and visceral components with characteristic hyperinflammation, antigen presentation dysregulation, production of autoantibodies by B cell. (E) Autoantibodies produced by aberrant B cells against metabolically important organelles including the pancreas potentially predispose individuals to early onset of diabetes, and (F) autoimmune cases of anti‐IFN antibodies dampening the early innate immune response against infection providing opportunities to latent/opportunistic infections such as herpes virus.
Box 2 – Completed clinical trials (as of June 2024) investigating drug interventions for post‐acute sequelae of coronavirus disease 2019 (PASC)*
National clinical trial number |
Study title |
Brief summary |
Interventions |
||||||||||||
|
|||||||||||||||
NCT05047952 |
Vortioxetine for Post‐COVID‐19 Condition |
To evaluate vortioxetine, an antidepressant with established pro‐cognitive properties, for the treatment of cognitive deficits that develop during or after an infection consistent with COVID‐19 |
Vortioxetine v placebo |
||||||||||||
NCT05472090 |
Evaluate the efficacy and safety of TNX‐102 SL in Patients With Multi‐Site Pain Associated With Post‐Acute Sequelae of SARS‐CoV‐2 Infection |
To evaluate the efficacy and safety of TNX‐102 SL 5.6 mg (2 × 2.8 mg tablets) taken once daily at bedtime for the management of multisite pain associated with PASC |
TNX‐102 SL v placebo |
||||||||||||
NCT03554265 |
Brain and Gut Plasticity in Mild TBI or Post‐acute COVID Syndrome Following Growth Hormone Therapy |
This study aims to treat patients with mild traumatic brain injury (mTBI) or PASC who exhibit abnormal growth hormone levels, using hormone therapy for 6 or 9 months respectively |
Somatropin |
||||||||||||
NCT04871815 |
Effects of Sodium Pyruvate Nasal Spray in COVID‐19 Long Haulers |
This study aims to evaluate the effects of N115 (sodium pyruvate nasal spray) on the symptoms associated with PASC |
Sodium pyruvate nasal spray |
||||||||||||
NCT04944121 |
Phase 2 Study of RSLV‐132 in Subjects With Long COVID |
The purpose of this study is to assess the efficacy (decrease in profound fatigue), safety and pharmacokinetics of RSLV‐132 in subjects with PASC |
RSLV‐132 v sodium chloride 0.9% |
||||||||||||
NCT05592418 |
Study to Evaluate the Efficacy and Safety of Ampligen in Patients With Post‐COVID Conditions |
The purpose of this study is to assess the efficacy and safety of Ampligen (AIM Immunotech) administered twice per week by intravenous infusions in subjects experiencing the post‐COVID‐19 condition of fatigue |
Rintatolimod v placebo |
||||||||||||
NCT05074888 |
Efficacy and Safety of Prospekta in the Treatment of Post‐COVID‐19 Asthenia |
The objective of this study is to evaluate the efficacy and safety of Prospekta (Materia Medica) in the treatment of asthenia in patients after COVID‐19 |
Prospekta v placebo |
||||||||||||
NCT05052307 |
A Real‐world Evidence Study of BNT162b2 mRNA COVID‐19 Vaccine in Brazil |
This study aims to estimate the real‐world effectiveness of the Pfizer–BioNTech BNT162b2 mRNA vaccine against symptomatic SARS‐CoV‐2 infection and its outcomes following a mass vaccination effort in Toledo, Southern Brazil |
Pfizer–BioNTech BNT162b2 mRNA COVID‐19 vaccine, CoronaVac COVID‐19 vaccine, ChAdOx1 nCoV‐19 COVID‐19 vaccine, Ad26.COV2.S COVID‐19 vaccine |
||||||||||||
NCT05576662 |
Paxlovid for Treatment of Long COVID |
The purpose of this study is to compare whether being treated with Paxlovid (Pfizer) (nirmatrelvir plus ritonavir) for 15 days works better than being treated with placebo (plus ritonavir) to reduce severe symptoms of long COVID |
Nirmatrelvir plus ritonavir v placebo plus ritonavir |
||||||||||||
NCT04809974 |
Clinical Trial of Niagen to Examine Recovery in People With Persistent Cognitive and Physical Symptoms After COVID‐19 Illness (Long‐COVID) |
The study will assess whether Niagen a safe dietary supplement, improves recovery of COVID‐19‐related symptoms in individuals who were infected at least 2 months before study entry |
Niagen |
||||||||||||
NCT04604704 |
Pilot Study Into LDN and NAD+ for Treatment of Patients With Post‐COVID‐19 Syndrome |
Pilot study into low dose naltrexone (LDN) and nicotinamide adenine dinucleotide (NAD+) for treatment of patients with PASC |
Naltrexone v dietary supplement: NAD+ |
||||||||||||
NCT04997395 |
Feasibility of Cannabidiol for the Treatment of Long COVID |
The aim is to assess the feasibility of recruiting and retaining individuals diagnosed with PASC into a treatment trial of medicinal cannabis, as well as assessing the safety and tolerability of dominant medicinal cannabis in this population |
MediCabilis Cannabis sativa 50 (Bod Australia) |
||||||||||||
NCT06383819 |
Efficacy and Safety of Longidaza for the Treatment of Patients With Residual Changes in the Lungs After COVID‐19 |
The goal of this clinical study is to evaluate the efficacy and safety of Longidaza (Petrovax), lyophilizate for preparation of solution for injection, at a dose of 3000 IU compared with placebo in the treatment of adult patients with residual changes in the lungs after COVID‐19 infection |
Longidaza v placebo |
||||||||||||
NCT05618587 |
Effect of Lithium Therapy on Long COVID Symptoms |
This study will assess the effects of low dose lithium on several different symptoms experienced by patients with PASC |
Lithium v placebo |
||||||||||||
NCT05633407 |
Efficacy and Safety Study of Efgartigimod in Adults With Post‐COVID‐19 POTS |
The study aims to investigate the safety, tolerability, efficacy, pharmacodynamics, pharmacokinetics, and immunogenicity of efgartigimod compared with placebo in participants with post‐COVID‐19 postural orthostatic tachycardia syndrome (POTS) (post‐COVID‐19 POTS) |
Efgartigimod v placebo |
||||||||||||
NCT05152849 |
Efficacy, Safety, and Tolerability of AXA1125 in Fatigue After COVID‐19 Infection |
This study will compare the effects of AXA1125 (Axcella Health), an orally active mixture of amino acids, compared with placebo, on improving muscle function (metabolism) following moderate exercise in subjects with fatigue‐predominant PASC as well as the safety and tolerability of AXA1125. Subjects will take one dose of AXA1125 or a placebo twice per day for 28 days |
AXA1125 v placebo |
||||||||||||
|
|||||||||||||||
COVID‐19 = coronavirus disease 2019. * Trial results are not yet available. |
Box 3 – Summary of research gaps in hypothesised post‐acute sequelae of coronavirus disease 2019 (PASC) mechanisms
COVID‐19 mechanism |
Research gaps |
||||||||||||||
|
|||||||||||||||
Dysfunctional neurological signalling |
|
||||||||||||||
Gut dysbiosis |
|
||||||||||||||
Immunothrombosis |
|
||||||||||||||
Immune dysregulation |
|
||||||||||||||
|
|
||||||||||||||
|
|||||||||||||||
COVID‐19 = coronavirus disease 2019; EBV = Epstein–Barr virus; IFN = interferon; SARS‐CoV‐2 = severe acute respiratory syndrome coronavirus 2. |
Provenance: Not commissioned; externally peer reviewed.
- Anurag Adhikari1,2
- Janesha Maddumage1
- Emily M Eriksson3
- Sarah J Annesley4
- Victoria A Lawson5
- Vanessa L Bryant3,6,7
- Stephanie Gras1,8
- 1 La Trobe Institute for Molecular Science, La Trobe University, Melbourne, VIC
- 2 Kathmandu Research Institute for Biological Sciences, Lalitpur, Nepal
- 3 Walter and Eliza Hall Institute of Medical Research, Melbourne, VIC
- 4 La Trobe University, Melbourne, VIC
- 5 Peter Doherty Institute for Infection and Immunity, University of Melbourne, Melbourne, VIC
- 6 Royal Melbourne Hospital, Melbourne, VIC
- 7 University of Melbourne, Melbourne, VIC
- 8 Monash University, Melbourne, VIC
Open access:
Open access publishing facilitated by La Trobe University, as part of the Wiley ‐ La Trobe University agreement via the Council of Australian University Librarians.
No relevant disclosures.
- 1. World Health Organization. Post COVID‐19 condition (long COVID) [website]. WHO, 2022. https://www.who.int/europe/news‐room/fact‐sheets/item/post‐covid‐19‐condition (viewed Aug 2023).
- 2. Australian Institute of Health and Welfare. Long COVID in Australia — a review of the literature [Cat. No. PHE 318]. Canberra: AIHW, 2022. https://www.aihw.gov.au/reports/covid‐19/long‐covid‐in‐australia‐a‐review‐of‐the‐literature/summary (viewed Aug 2024).
- 3. Woodrow M, Carey C, Ziauddeen N, et al. Systematic review of the prevalence of long COVID. Open Forum Infect Dis 2023; 10: ofad233.
- 4. Department of Health, Victorian Government. Victorian Experience with COVID‐19 Health Survey. https://vahi.vic.gov.au/news/conference‐wrap‐firsthand‐experiences‐drive‐efforts‐improve‐outcomes‐people‐long‐covid (viewed Aug 2023).
- 5. Ceban F, Ling S, Lui LMW, et al. Fatigue and cognitive impairment in post‐COVID‐19 syndrome: a systematic review and meta‐analysis. Brain Behav Immun 2022; 101: 93‐135.
- 6. Al‐Aly Z, Bowe B, Xie Y. Long COVID after breakthrough SARS‐CoV‐2 infection. Nat Med 2022; 28: 1461‐1467.
- 7. World Health Organization. WHO COVID‐19 dashboard: number of COVID‐19 cases reported to WHO. https://data.who.int/dashboards/covid19/cases (viewed Oct 2023).
- 8. Mansell V, Hall Dykgraaf S, Kidd M, Goodyear‐Smith F. Long COVID and older people. Lancet Healthy Longev 2022; 3: e849‐e854.
- 9. Tsampasian V, Elghazaly H, Chattopadhyay R, et al. Risk factors associated with post‐COVID‐19 condition: a systematic review and meta‐analysis. JAMA Intern Med 2023; 183: 566‐580.
- 10. Department of Health and Aged Care, Australian Government. COVID‐19 reporting. https://www.health.gov.au/health‐alerts/covid‐19/weekly‐reporting (viewed Oct 2023).
- 11. Malesevic S, Sievi NA, Baumgartner P, et al. Impaired health‐related quality of life in long‐COVID syndrome after mild to moderate COVID‐19. Sci Rep 2023; 13: 7717.
- 12. Higgins V, Sohaei D, Diamandis EP, Prassas I. COVID‐19: from an acute to chronic disease? Potential long‐term health consequences. Crit Rev Clin Lab Sci 2021; 58: 297‐310.
- 13. Davis HE, McCorkell L, Vogel JM, Topol EJ. Long COVID: major findings, mechanisms and recommendations. Nat Rev Microbiol 2023; 21: 133‐146.
- 14. Peluso MJ, Deveau TM, Munter SE, et al. Chronic viral coinfections differentially affect the likelihood of developing long COVID. J Clin Invest 2023; 133: e163669.
- 15. Monje M, Iwasaki A. The neurobiology of long COVID. Neuron 2022; 110: 3484‐3496.
- 16. Jammoul M, Naddour J, Madi A, et al. Investigating the possible mechanisms of autonomic dysfunction post‐COVID‐19. Auton Neurosci 2023; 245: 103071.
- 17. Pellegrini L, Albecka A, Mallery DL, et al. SARS‐CoV‐2 infects the brain choroid plexus and disrupts the blood‐CSF barrier in human brain organoids. Cell Stem Cell 2020; 27: 951‐961.
- 18. Song E, Bartley CM, Chow RD, et al. Divergent and self‐reactive immune responses in the CNS of COVID‐19 patients with neurological symptoms. Cell Rep Med 2021; 2: 100288.
- 19. Andrews MG, Mukhtar T, Eze UC, et al. Tropism of SARS‐CoV‐2 for human cortical astrocytes. Proc Natl Acad Sci USA 2022; 119: e2122236119.
- 20. Jeong GU, Lyu J, Kim KD, et al. SARS‐CoV‐2 infection of microglia elicits proinflammatory activation and apoptotic cell death. Microbiol Spectr 2022; 10: e0109122.
- 21. Beckman D, Bonillas A, Diniz GB, et al. SARS‐CoV‐2 infects neurons and induces neuroinflammation in a non‐human primate model of COVID‐19. Cell Rep 2022; 41: 111573.
- 22. Martínez‐Mármol R, Giordano‐Santini R, Kaulich E, et al. SARS‐CoV‐2 infection and viral fusogens cause neuronal and glial fusion that compromises neuronal activity. Sci Adv 2023; 9: eadg2248.
- 23. Fernández‐Castañeda A, Lu P, Geraghty AC, et al. Mild respiratory COVID can cause multi‐lineage neural cell and myelin dysregulation. Cell 2022; 185: 2452‐2468.
- 24. Nieuwland JM, Nutma E, Philippens I, et al. Longitudinal positron emission tomography and postmortem analysis reveals widespread neuroinflammation in SARS‐CoV‐2 infected rhesus macaques. J Neuroinflammation 2023; 20: 179.
- 25. Wang EY, Mao T, Klein J, et al. Diverse functional autoantibodies in patients with COVID‐19. Nature 2021; 595: 283‐288.
- 26. Kong W, Montano M, Corley MJ, et al. Neuropilin‐1 mediates SARS‐CoV‐2 infection of astrocytes in brain organoids, inducing inflammation leading to dysfunction and death of neurons. mBio 2022; 13: e0230822.
- 27. Lavi Y, Vojdani A, Halpert G, et al. Dysregulated levels of circulating autoantibodies against neuronal and nervous system autoantigens in COVID‐19 patients. Diagnostics (Basel) 2023; 13: 687.
- 28. Garber C, Soung A, Vollmer LL, et al. T cells promote microglia‐mediated synaptic elimination and cognitive dysfunction during recovery from neuropathogenic flaviviruses. Nat Neurosci 2019; 22: 1276‐1288.
- 29. Hanson BA, Visvabharathy L, Ali ST, et al. Plasma biomarkers of neuropathogenesis in hospitalized patients with COVID‐19 and those with postacute sequelae of SARS‐CoV‐2 infection. Neurol Neuroimmunol Neuroinflamm 2022; 9: e1151.
- 30. Zhang H, Li HB, Lyu JR, et al. Specific ACE2 expression in small intestinal enterocytes may cause gastrointestinal symptoms and injury after 2019‐nCoV infection. Int J Infect Dis 2020; 96: 19‐24.
- 31. Lee S, Yoon GY, Myoung J, et al. Robust and persistent SARS‐CoV‐2 infection in the human intestinal brush border expressing cells. Emerg Microbes Infect 2020; 9: 2169‐2179.
- 32. Settanni CR, Ianiro G, Ponziani FR, et al. COVID‐19 as a trigger of irritable bowel syndrome: A review of potential mechanisms. World J Gastroenterol 2021; 27: 7433‐7445.
- 33. Teixeira PC, Dorneles GP, Santana Filho PC, et al. Increased LPS levels coexist with systemic inflammation and result in monocyte activation in severe COVID‐19 patients. Int Immunopharmacol 2021; 100: 108125.
- 34. Lau WL, Kalantar‐Zadeh K, Vaziri ND. The gut as a source of inflammation in chronic kidney disease. Nephron 2015; 130: 92‐98.
- 35. Giron LB, Peluso MJ, Ding J, et al. Markers of fungal translocation are elevated during post‐acute sequelae of SARS‐CoV‐2 and induce NF‐κB signaling. JCI Insight 2022; 7: e160989.
- 36. Schultheiss C, Willscher E, Paschold L, et al. The IL‐1β, IL‐6, and TNF cytokine triad is associated with post‐acute sequelae of COVID‐19. Cell Rep Med 2022; 3: 100663.
- 37. Hobby GP, Karaduta O, Dusio GF, et al. Chronic kidney disease and the gut microbiome. Am J Physiol Renal Physiol 2019; 316: F1211‐F1217.
- 38. Pergolizzi J, LeQuang JAK, Breve F, et al. Exploring the implications of new‐onset diabetes in COVID‐19: a narrative review. Cureus 2023; 15: e33319.
- 39. Cortes GM, Marcialis MA, Bardanzellu F, et al. Inflammatory bowel disease and COVID‐19: how microbiomics and metabolomics depict two sides of the same coin. Front Microbiol 2022; 13: 856165.
- 40. Assante G, Williams R, Youngson NA. Is the increased risk for MAFLD patients to develop severe COVID‐19 linked to perturbation of the gut‐liver axis? J Hepatol 2021; 74: 487‐488.
- 41. Guarnieri JW, Dybas JM, Fazelinia H, et al. Core mitochondrial genes are down‐regulated during SARS‐CoV‐2 infection of rodent and human hosts. Sci Transl Med 2023; 15: eabq1533.
- 42. Cortese M, Lee JY, Cerikan B, et al. Integrative imaging reveals SARS‐CoV‐2‐induced reshaping of subcellular morphologies. Cell Host Microbe 2020; 28: 853‐866.
- 43. Motta CS, Torices S, da Rosa BG, et al. Human brain microvascular endothelial cells exposure to SARS‐CoV‐2 leads to inflammatory activation through NF‐κB non‐canonical pathway and mitochondrial remodeling. Viruses 2023; 15: 745.
- 44. Mando C, Savasi VM, Anelli GM, et al. Mitochondrial and oxidative unbalance in placentas from mothers with SARS‐CoV‐2 infection. Antioxidants (Basel) 2021; 10: 1517.
- 45. Lai AL, Freed JH. SARS‐CoV‐2 fusion peptide has a greater membrane perturbating effect than SARS‐CoV with highly specific dependence on Ca2. J Mol Biol 2021; 433: 166946.
- 46. Wood E, Hall KH, Tate W. Role of mitochondria, oxidative stress and the response to antioxidants in myalgic encephalomyelitis/chronic fatigue syndrome: a possible approach to SARS‐CoV‐2 “long‐haulers”? Chronic Dis Transl Med 2021; 7: 14‐26.
- 47. Vernon SD, Whistler T, Cameron B, et al. Preliminary evidence of mitochondrial dysfunction associated with post‐infective fatigue after acute infection with Epstein Barr virus. BMC Infect Dis 2006; 6: 15.
- 48. Laura M, Sarah W, Benjamin G, et al. Plasma mediators in patients with severe COVID‐19 cause lung endothelial barrier failure. Eur Respir J 2021; 57: 2002384.
- 49. Yong SJ. Long COVID or post‐COVID‐19 syndrome: putative pathophysiology, risk factors, and treatments. Infect Dis (Lond) 2021; 53: 737‐754.
- 50. Conway EM, Mackman N, Warren RQ, et al. Understanding COVID‐19‐associated coagulopathy. Nat Rev Immunol 2022; 22: 639‐649.
- 51. Wu C, Lu W, Zhang Y, et al. Inflammasome activation triggers blood clotting and host death through pyroptosis. Immunity 2019; 50: 1401‐1411.
- 52. Lazzaroni MG, Piantoni S, Masneri S, et al. Coagulation dysfunction in COVID‐19: the interplay between inflammation, viral infection and the coagulation system. Blood Rev 2021; 46: 100745.
- 53. Haffke M, Freitag H, Rudolf G, et al. Endothelial dysfunction and altered endothelial biomarkers in patients with post‐COVID‐19 syndrome and chronic fatigue syndrome (ME/CFS). J Transl Med 2022; 20: 138.
- 54. Østergaard L. SARS CoV‐2 related microvascular damage and symptoms during and after COVID‐19: consequences of capillary transit‐time changes, tissue hypoxia and inflammation. Physiol Rep 2021; 9: e14726.
- 55. Swank Z, Senussi Y, Manickas‐Hill Z, et al. Persistent circulating severe acute respiratory syndrome coronavirus 2 spike is associated with post‐acute coronavirus disease 2019 sequelae. Clin Infect Dis 2023; 76: e487‐e490.
- 56. Lei Y, Zhang J, Schiavon CR, et al. SARS‐CoV‐2 spike protein impairs endothelial function via downregulation of ACE2. Circ Res 2021; 128: 1323‐1326.
- 57. Evans PC, Rainger GE, Mason JC, et al. Endothelial dysfunction in COVID‐19: a position paper of the ESC Working Group for Atherosclerosis and Vascular Biology, and the ESC Council of Basic Cardiovascular Science. Cardiovasc Res 2020; 116: 2177‐2184.
- 58. Kostov K. The causal relationship between endothelin‐1 and hypertension: focusing on endothelial dysfunction, arterial stiffness, vascular remodeling, and blood pressure regulation. Life (Basel) 2021; 11: 986.
- 59. Charfeddine S, Ibn Hadj Amor H, Jdidi J, et al. Long COVID 19 syndrome: is it related to microcirculation and endothelial dysfunction? Insights from TUN‐EndCOV study. Front Cardiovasc Med 2021; 8: 745758.
- 60. Kuchler T, Gunthner R, Ribeiro A, et al. Persistent endothelial dysfunction in post‐COVID‐19 syndrome and its associations with symptom severity and chronic inflammation. Angiogenesis 2023; 26: 547‐563.
- 61. Frishberg A, Kooistra E, Nuesch‐Germano M, et al. Mature neutrophils and a NF‐κB‐to‐IFN transition determine the unifying disease recovery dynamics in COVID‐19. Cell Rep Med 2022; 3: 100652.
- 62. Jukema BN, Smit K, Hopman MTE, et al. Neutrophil and eosinophil responses remain abnormal for several months in primary care patients with COVID‐19 disease. Front Allergy 2022; 3: 942699.
- 63. Pisareva E, Badiou S, Mihalovičová L, et al. Persistence of neutrophil extracellular traps and anticardiolipin auto‐antibodies in post‐acute phase COVID‐19 patients. J Med Virol 2023; 95: e28209.
- 64. Weinstock LB, Brook JB, Walters AS, et al. Mast cell activation symptoms are prevalent in long‐COVID. Int J Infect Dis 2021; 112: 217‐226.
- 65. Krysko O, Bourne JH, Kondakova E, et al. Severity of SARS‐CoV‐2 infection is associated with high numbers of alveolar mast cells and their degranulation. Front Immunol 2022; 13: 968981.
- 66. Patterson BK, Francisco EB, Yogendra R, et al. Persistence of SARS CoV‐2 S1 protein in CD16+ monocytes in post‐acute sequelae of COVID‐19 (PASC) up to 15 months post‐infection. Front Immunol 2021; 12: 746021.
- 67. Ryan FJ, Hope CM, Masavuli MG, et al. Long‐term perturbation of the peripheral immune system months after SARS‐CoV‐2 infection. BMC Med 2022; 20: 26.
- 68. Peluso MJ, Deitchman AN, Torres L, et al. Long‐term SARS‐CoV‐2‐specific immune and inflammatory responses in individuals recovering from COVID‐19 with and without post‐acute symptoms. Cell Rep 2021; 36: 109518.
- 69. Phetsouphanh C, Darley DR, Wilson DB, et al. Immunological dysfunction persists for 8 months following initial mild‐to‐moderate SARS‐CoV‐2 infection. Nat Immunol 2022; 23: 210‐216.
- 70. Wiech M, Chroscicki P, Swatler J, et al. Remodeling of T cell dynamics during long COVID is dependent on severity of SARS‐CoV‐2 infection. Front Immunol 2022; 13: 886431.
- 71. Kim JYH, Ragusa M, Tortosa F, et al. Viral reactivations and co‐infections in COVID‐19 patients: a systematic review. BMC Infect Dis 2023; 23: 259.
- 72. Santopaolo M, Gregorova M, Hamilton F, et al. Prolonged T‐cell activation and long COVID symptoms independently associate with severe COVID‐19 at 3 months. Elife 2023; 12: e85009.
- 73. Bull‐Otterson L, K SBSST, Gray BSAS, Harris AM. Post‐COVID conditions among adult COVID‐19 survivors aged 18–64 and ≥ 65 years — United States, March 2020 – November 2021. MMWR Morb Mortal Wkly Rep 2022; 71: 713‐717.
- 74. Varnai R, Molnar T, Zavori L, et al. Serum level of anti‐nucleocapsid, but not anti‐spike antibody, is associated with improvement of long COVID symptoms. Vaccines (Basel) 2022; 10: 165.
- 75. Augustin M, Schommers P, Stecher M, et al. Post‐COVID syndrome in non‐hospitalised patients with COVID‐19: a longitudinal prospective cohort study. Lancet Reg Health Eur 2021; 6: 100122.
- 76. Fedorchenko Y, Zimba O. Long COVID in autoimmune rheumatic diseases. Rheumatol Int 2023; 43: 1197‐1207.
- 77. Grubišić B, Švitek L, Ormanac K, et al. Molecular mechanisms responsible for diabetogenic effects of COVID‐19 infection‐induction of autoimmune dysregulation and metabolic disturbances. Int J Mol Sci 2023; 24: 11576.
- 78. Zhang T, Mei Q, Zhang Z, et al. Risk for newly diagnosed diabetes after COVID‐19: a systematic review and meta‐analysis. BMC Med 2022; 20: 444.
- 79. Wu CT, Lidsky PV, Xiao Y, et al. SARS‐CoV‐2 infects human pancreatic β cells and elicits β cell impairment. Cell Metab 2021; 33: 1565‐1576.
- 80. Baimukhamedov C, Barskova T, Matucci‐Cerinic M. Arthritis after SARS‐CoV‐2 infection. Lancet Rheumatol 2021; 3: e324‐e325.
- 81. Ramachandran L, Dontaraju VS, Troyer J, Sahota J. New onset systemic lupus erythematosus after COVID‐19 infection: a case report. AME Case Rep 2022; 6: 14.
- 82. Bastard P, Gervais A, Le Voyer T, et al. Autoantibodies neutralizing type I IFNs are present in ~4% of uninfected individuals over 70 years old and account for ~20% of COVID‐19 deaths. Sci Immunol 2021; 6: eabl4340.
- 83. Matyushkina D, Shokina V, Tikhonova P, et al. Autoimmune effect of antibodies against the SARS‐CoV‐2 nucleoprotein. Viruses 2022; 14: 1141.
- 84. Bastard P, Rosen LB, Zhang Q, et al. Autoantibodies against type I IFNs in patients with life‐threatening COVID‐19. Science 2020; 370: eabd4585.
- 85. Solanich X, Rigo‐Bonnin R, Gumucio VD, et al. Pre‐existing autoantibodies neutralizing high concentrations of type I Interferons in almost 10% of COVID‐19 patients admitted to intensive care in Barcelona. J Clin Immunol 2021; 41: 1733‐1744.
- 86. Su Y, Yuan D, Chen DG, et al. Multiple early factors anticipate post‐acute COVID‐19 sequelae. Cell 2022; 185: 881‐895.
- 87. Wang F, Ma Y, Xu S, et al. Prevalence and risk of COVID‐19 in patients with rheumatic diseases: a systematic review and meta‐analysis. Clin Rheumatol 2022; 41: 2213‐2223.
- 88. Panayiotis GV, Eleni M, Haris A, et al. Autoantibodies related to systemic autoimmune rheumatic diseases in severely ill patients with COVID‐19. Ann Rheum Dis 2020; 79: 1661‐1663.
- 89. Klein J, Wood J, Jaycox J, et al. Distinguishing features of long COVID identified through immune profiling. Nature 2023; 623: 139‐148.
Summary